Have you ever marveled at the harmonious sound produced by a tuning fork vibrating in response to another tuning fork of the same frequency? This captivating occurrence is an example of a phenomenon known as resonance. Resonance is not limited to the world of acoustics; it also plays a significant role in the realm of engineering and structural dynamics. In this blog post, we will delve into the fascinating concept of structural resonance, uncovering its principles, effects, and practical implications.
What is Resonance?
Resonance is a phenomenon that occurs when a system vibrates at its natural frequency or a multiple thereof in response to an external force or vibration. In simpler terms, resonance occurs when an object or system “resonates” or vibrates with a greater amplitude in response to a specific frequency of external stimulus. When the forcing frequency matches the system frequency, resonance occurs and vibration amplitude becomes very high.
Types of Resonance
Resonance is a fascinating phenomenon that can occur in various contexts, each with its own distinct characteristics and effects. There are several types of resonance, each with its unique attributes. Here are some of the most common types:
Mechanical Resonance:
This type of resonance involves the vibration of mechanical systems. It can occur in structures, machinery, and other physical systems. Mechanical resonance can lead to the amplification of vibrations and potentially result in damage or failure. Examples include bridges swaying in response to wind or a guitar string vibrating when plucked.
Acoustic Resonance:
Acoustic resonance occurs in sound waves and is particularly evident in enclosed spaces. When sound waves bounce off surfaces and interfere constructively, they can create areas of amplified sound known as resonant cavities. Musical instruments like string instruments and wind instruments rely on acoustic resonance to produce their unique sounds.
Electromagnetic Resonance:
This type of resonance is observed in electromagnetic waves, such as radio waves, microwaves, and light waves. Resonant structures can absorb or transmit specific frequencies with greater efficiency, as seen in antennas or microwave ovens.
Electrical Resonance:
Electrical circuits can exhibit resonance when the frequency of an external electrical signal matches the circuit’s natural frequency. This can lead to amplified electrical currents and voltages in the circuit. Resonance is commonly encountered in applications like radio tuning circuits and power distribution networks.
Atomic and Molecular Resonance:
In the realm of quantum physics, atoms, and molecules can absorb or emit electromagnetic radiation at specific resonant frequencies. This phenomenon is crucial in fields such as spectroscopy, where researchers use resonant interactions to study the properties of matter.
Nuclear Magnetic Resonance (NMR):
NMR is a technique used in chemistry, biology, and medicine to study the properties of atomic nuclei. It involves placing a sample in a strong magnetic field and applying radiofrequency pulses to induce resonance in the nuclei. NMR is the basis for technologies like magnetic resonance imaging (MRI).
Structural Resonance:
Structural resonance occurs when the natural frequency of a physical structure matches an external force’s frequency. This can lead to amplified vibrations and oscillations, potentially causing damage or failure in the structure.
Fluid Dynamic Resonance:
Fluids, such as air or liquids, can also exhibit resonance. This is observed in scenarios like the singing wine glass phenomenon, where sound waves cause vibrations in the glass due to matching frequencies.
Biological Resonance:
Biological systems, such as the human body, can also exhibit resonance. For instance, when a singer’s voice matches the resonant frequency of a glass, it can shatter. In medicine, ultrasonic waves are used for imaging and therapy based on biological tissue’s resonant properties.
Cavity Resonance:
Enclosed spaces, such as rooms, can exhibit resonance at specific frequencies, leading to an increase in sound levels at those frequencies. This phenomenon is significant in architectural acoustics and audio engineering.
These various types of resonance showcase the universal nature of the phenomenon and its applications across different scientific and engineering disciplines. While resonance can lead to beneficial effects in certain contexts, understanding and managing it is crucial to avoid unwanted consequences, especially when dealing with structural or mechanical systems.
Examples of Resonance
- Swinging on a Swing: Pushing a swing at the right time and rhythm allows it to swing higher and higher. This is an example of resonance in a mechanical system.
- Tuning Forks: Striking a tuning fork and placing it close to another tuning fork with the same frequency causes the second fork to vibrate as well, producing a clear, sustained sound. This demonstrates acoustic resonance.
- Radio Tuning: Adjusting a radio tuner to a specific frequency results in better reception of that radio station due to electromagnetic resonance in the tuner circuit.
- Breaking a Wine Glass: Singing at a specific pitch can cause a wine glass to shatter due to acoustic resonance between the sound waves and the glass’s natural frequency.
- Bridges and Wind: Strong winds can cause bridges to sway if the wind’s frequency matches the bridge’s natural frequency, leading to structural resonance.
Resonance can have both positive and negative effects, depending on the context. While it is harnessed in musical instruments and various technologies, it can also lead to undesirable consequences, such as equipment failure, structural damage, or reduced efficiency. In engineering and design, understanding and managing resonance are crucial to ensuring the safe and effective operation of systems and structures.
What is Structural Resonance?
Structural resonance is a phenomenon that occurs when a dynamic force or vibration applied to a structure matches its natural frequency. Similar to how a singer can shatter a glass by producing the glass’s natural resonant frequency, a structure can experience amplified vibrations when exposed to external forces at its resonant frequency. This interaction between the external force and the structure’s natural frequency can lead to substantial oscillations, which may result in undesirable consequences.
Principles of Structural Resonance
To understand structural resonance better, let’s break down the underlying principles:
Natural Frequency: Every physical structure has a characteristic natural frequency at which it naturally vibrates. This frequency is determined by the structure’s material properties, geometry, and boundary conditions.
Excitation Frequency: When an external force or vibration is applied to a structure, it contains a range of frequencies. If the excitation frequency matches or is close to the structure’s natural frequency, resonance can occur.
Amplification of Vibrations: When resonance occurs, the structure’s vibrations are amplified, often resulting in increased displacement and strain. This amplification can lead to structural damage or failure if not properly managed.
Energy Transfer: Resonance involves the transfer of energy between the external force and the system. Energy is absorbed from the external force and is stored as kinetic energy in the system’s vibrations.
Effects of Structural Resonance
The effects of structural resonance can be far-reaching and impactful. Some of the notable consequences include:
Increased Stress:
Amplified vibrations can subject the pump and its components to higher levels of stress and strain, potentially leading to premature wear, fatigue, or even mechanical failure. Over time, repeated exposure to resonant vibrations can lead to mechanical damage, such as loosening of bolts, misalignment of components, or even cracks in structural elements.
Fatigue and Damage:
Prolonged exposure to resonant vibrations can lead to material fatigue and structural damage. Over time, the repeated stress caused by resonant vibrations can weaken the structure, potentially leading to cracks, fractures, or other forms of degradation.
Reduced Performance:
Resonance can disrupt the normal functioning of mechanical systems and components, affecting their performance and efficiency. This is particularly crucial in industries such as aerospace, where precise and reliable performance is essential.
Catastrophic Failures:
In extreme cases, structural resonance can lead to catastrophic failures, especially if the amplified vibrations cause a critical component to malfunction or break. Such failures can have severe safety, economic, and environmental implications.
What is the Bode plot and what is its Importance in Structural Resonance?
A Bode plot is a graphical representation used in engineering and science to analyze the frequency response of a system. It consists of two plots: one representing the system’s gain (amplitude response) and the other representing its phase shift (phase response) as a function of frequency. Bode plots (Fig. 1) are particularly useful for understanding how a system responds to different frequencies and can provide insights into resonance, stability, and overall system behavior.
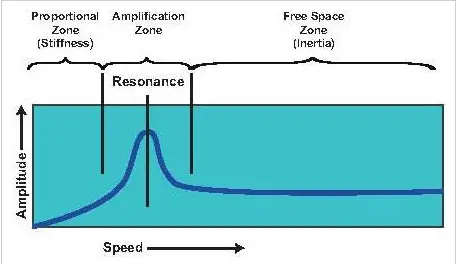
Significance of Bode Plot in Structural Resonance
The importance of Bode plots in the context of structural resonance lies in their ability to predict and analyze the behavior of mechanical systems subjected to varying frequencies of external forces or vibrations. Here’s how Bode plots are relevant to understanding and managing structural resonance:
Frequency Analysis:
Bode plots allow engineers to visualize how a system responds to different frequencies. In the case of structural resonance, it helps identify the system’s natural frequencies and the frequencies at which resonant behavior may occur.
Resonance Identification:
Bode plots can clearly show where peaks in the amplitude response occur. These peaks correspond to the system’s resonant frequencies. By analyzing the Bode plot, engineers can identify the frequencies at which resonance might occur.
Amplitude Magnification:
Bode plots provide information about the magnitude of the system’s response to different frequencies. In the presence of resonance, the amplitude response can significantly magnify at or near the system’s natural frequencies, indicating the potential for amplified vibrations and oscillations.
Phase Shift:
The phase response shown in Bode plots helps engineers understand the phase relationship between input and output signals. This information is crucial for predicting how vibrations might interact with the structure, potentially leading to reinforcement or cancellation effects.
Stability Assessment:
In the context of resonance, stability is a concern. Bode plots can help engineers assess the stability of a system by analyzing the phase margin and gain margin. If a system becomes unstable due to resonance, it can lead to unpredictable and potentially harmful behavior.
Design and Mitigation:
Bode plots guide the design process by allowing engineers to adjust system parameters to avoid resonant frequencies or implement damping measures to mitigate resonance effects.
Dynamic Analysis:
Structural dynamics involve the study of how structures respond to dynamic loads. Bode plots help engineers predict and understand the dynamic behavior of structures, which is crucial for preventing failures due to resonance.
Validation and Testing:
Bode plots can be used to validate simulation results against real-world testing. By comparing predicted and actual frequency responses, engineers can refine their models and ensure accurate predictions of resonance behavior.
In summary, Bode plots provide a powerful tool for analyzing the frequency response of mechanical systems, which is fundamental to understanding and managing structural resonance. They allow engineers to identify resonant frequencies, assess amplitude magnification, analyze phase relationships, and make informed decisions about design modifications and mitigation strategies to ensure the safety and reliability of structures subjected to dynamic forces.
Managing and Mitigating Resonance
Engineers and designers employ various strategies to manage and mitigate the risks associated with structural resonance:
Vibration Isolation:
Implementing vibration isolation measures, such as installing resilient mounts or vibration isolators between the vibration source and the system, can help reduce the transmission of external vibrations to the system.
Frequency Analysis:
By conducting thorough frequency analysis during the design phase, engineers can identify potential resonance points and adjust the structure’s properties to avoid critical resonant frequencies.
Damping Systems:
Introducing damping systems, such as vibration absorbers or isolators, can help dissipate energy and reduce the impact of resonant vibrations.
Tuned Mass Dampers:
These devices consist of a mass attached to the structure via a spring and a damper. They are designed to counteract the effects of resonance by introducing out-of-phase vibrations that cancel out the resonant response.
Structural Modifications:
Altering a structure’s geometry, material properties, or boundary conditions can shift its natural frequencies away from potential excitation frequencies.
Proper Installation of Rotating Equipment:
Ensuring proper installation and alignment of the pump and other rotating equipment components can minimize the likelihood of resonance occurring in pump, compressor, or turbine systems.
What is Damping and how it helps in managing Structural Resonance?
Damping refers to the dissipation of energy from a vibrating or oscillating system over time. It is a crucial concept in engineering and physics, as it plays a significant role in controlling and managing the effects of vibrations, including structural resonance. Damping helps to reduce the amplitude and duration of vibrations, preventing or minimizing the potentially damaging consequences of resonance.
In the context of structural resonance, damping is used to counteract the amplification of vibrations that can occur when an external force or vibration matches a structure’s natural frequency. By introducing damping mechanisms into a system, engineers can dissipate the energy of the resonant vibrations, effectively reducing their impact. Here’s how damping helps in managing structural resonance:
- Energy Dissipation: Damping mechanisms, such as dampers or absorbers, convert the kinetic energy of the vibrating system into heat or other forms of energy that is dissipated into the environment. This reduces the amount of energy available to sustain resonant vibrations.
- Reduction in Amplitude: Damping reduces the amplitude of vibrations, preventing the system from reaching large displacement levels that could lead to structural damage or failure.
- Shift in Resonant Frequencies: Damping can alter a system’s natural frequencies, shifting them away from potential excitation frequencies. This makes it less likely for resonance to occur even when external forces are present.
- Stabilization: Damping can help stabilize a system that might otherwise become unstable due to resonance. Uncontrolled resonance can lead to unpredictable and dangerous behavior, while damping helps maintain stability.
- Broadened Frequency Response: Damping can broaden the system’s frequency response, making it less sensitive to variations in frequency. This is particularly useful in environments where the frequency of external forces can vary.
- Vibration Control: Damping systems can be actively controlled to adapt to changing conditions. This allows engineers to fine-tune damping levels based on real-time data and external factors.
- Minimization of Fatigue: By reducing the amplitude of vibrations, damping helps minimize fatigue and wear on structural components, increasing the longevity of the system.
Common damping mechanisms used to manage structural resonance include:
- Viscous Damping: This involves introducing a viscous fluid or material that generates resistance to motion. As the system vibrates, the fluid’s viscosity dissipates energy, reducing vibration amplitudes.
- Structural Damping: Materials with inherent damping properties, such as certain rubber compounds, can be used to dampen vibrations.
- Tuned Mass Dampers: These devices consist of a mass attached to a structure via a spring and a damping mechanism. By adjusting the parameters of the damper, engineers can control the amount of damping introduced to the system.
- Vibration Absorbers: Similar to tuned mass dampers, vibration absorbers are designed to dissipate energy and reduce vibration amplitudes.
In summary, damping is a critical tool in managing structural resonance by dissipating energy, reducing vibrations, and stabilizing systems. By introducing damping mechanisms, engineers can effectively control and mitigate the potentially harmful effects of resonance in various engineering applications.
Conclusion
Structural resonance is a captivating yet potentially hazardous phenomenon that underscores the intricate relationship between external forces and the inherent properties of physical structures. By understanding its principles and effects, engineers and designers can make informed decisions to prevent, manage, and mitigate the risks associated with resonance. As technology and engineering continue to advance, our ability to predict, control, and harness structural resonance will play a pivotal role in creating safer, more efficient, and more resilient structures that withstand the test of time and external forces.